Main Content
New HHMI Investigators
By: Nicole Kresge
Illustration by Barry Falls.
As a teenager, Doris Tsao picked up Immanuel Kant’s Critique of Pure Reason, an investigation into the origin of human knowledge. The book is not an easy read. Tsao admits she made it through only the first 60 pages, but even that brief dip into Kant’s writings made a huge impression on her. Already set on a career in science, Tsao had her eyes opened by the book to the mysteries of the brain and spatial perception. While still in high school, she settled on the scientific problem she would devote her life to: How does the brain translate photons of light into recognizable objects?
Science is filled with questions like Tsao’s. How does the brain generate consciousness? How did life begin? How does a protein’s sequence determine its shape? Some scientists, like Tsao, find their questions at a young age. Others stumble upon them later in life. Regardless of their genesis, such questions are the force that pushes scientific exploration forward. Discovery doesn’t happen without inquiry, and every experiment starts with a question.
This past May, HHMI announced that Tsao and 25 other talented scientists would be given an initial five years of freedom to pursue the scientific questions that keep them up at night. The Institute has committed $153 million to support this newest cohort of HHMI investigators. Chosen from among 894 candidates, the researchers hail from 19 institutions and represent a variety of disciplines, ranging from computational biology to biochemistry to neuroscience. And with them, they bring a diverse array of problems they hope to solve.
Six Golden Eggs
Twenty years after dipping into Kant, Tsao, now at the California Institute of Technology, is still trying to get to the bottom of how the brain represents visual objects. Her dream, she says, is to understand the visual system with the same mathematical clarity that physicists use to understand the universe.
“One reason I’m so interested in object perception is that objects are essentially information folders,” she explains. “The contents of these folders are read in dozens and dozens of visual areas in the brain, and I’m trying to understand exactly how this is organized.”
Tsao made a major step toward cracking the brain’s filing system in 2006, when she was a postdoctoral fellow at Harvard Medical School. She and Harvard electrophysiologist Winrich Freiwald combined two of the most important tools in neuroscience – brain imaging and single neuron recordings – to reveal areas of the monkey brain whose sole purpose is recognizing faces. First, the pair used functional magnetic resonance imaging to get a bird’s-eye view of the brain and pinpoint the areas – six in all – that saw increased blood flow as monkeys viewed pictures of faces. Then, during what Tsao calls one of the most exciting days of her life, they probed one of those areas of the brain with electrodes and discovered that almost every single neuron in the patch fired only in response to faces. The same was true for the other five areas. These “face patches,” as Tsao named them, were the first concrete evidence that the primate brain operates something akin to a face-processing machine.
“These patches are like a half-dozen golden eggs, and we’re trying to understand how each one is processing faces in detail,” says Tsao. She’s only just scratched the surface, but it’s clear that the patches are working together to recognize and discriminate among faces. Each face patch has its own task – for example, some respond to certain characteristics like amount of hair or iris size, while others are in charge of recognizing faces at specific views, such as the front or side.
Next, Tsao wants to figure out how the brain takes bits of information – like facial features – and integrates them into a perception of a whole object. To this end, Tsao has recently embarked on a collaborative project with her mathematician father, Thomas Tsao. They’re working on a mathematical theory to solve one of the greatest problems in vision – the invariance problem, or how objects can be recognized despite changes in appearance due to perspective.
Radical Research
Squire Booker’s passion lies at the opposite end of the spectrum from Tsao’s. While Tsao is after the big picture of brain function, Booker is fascinated by the atomic details of chemical reactions. The origins of his research were not quite as deliberate as Tsao’s, either.
“When I was in college, I liked a lot of different areas of science, and I wanted to be able to do a little bit of everything,” he explains. “But one of my professors at Austin College told me, ‘If you want to do research, you’re going to have to choose. You’re going to have to decide what you want to be.’” Booker chose enzymology, a discipline that incorporates everything from analytical chemistry to bioinformatics. “I’m sort of a jack-of-all-trades,” he says. “Not necessarily a master of anything, but able to blend a lot of scientific disciplines into one.”
This cross-disciplined approach, combined with a nose for important research problems and a knack for clever experimental design, has allowed Booker to decipher the chemistry behind dozens of novel reactions in biology. In his lab at Pennsylvania State University, Booker studies a large family of proteins called radical S-adenosylmethionine (SAM) enzymes. The enzymes, which are found predominantly in anaerobic bacteria, harness the energy from an unstable form of S-adenosylmethionine to drive reluctant chemical reactions forward.
In true polymath fashion, Booker combines a variety of biochemical, enzymological, and structural tools with small-scale organic synthesis and fast-reaction kinetic methods to figure out what makes these radical reactions go.
One of those reactions is catalyzed lipoyl synthase, a radical SAM enzyme that adds sulfur atoms to octanoic acid inside cells. The resulting product, lipoic acid, is an important cofactor involved in energy metabolism and the breakdown of a number of different amino acids. “If you don’t have lipoic acid, you die,” Booker explains. In 2004, and most recently in 2014, he worked with Carsten Krebs, one of his colleagues at Penn State, to show that lipoyl synthase uses an unstable SAM molecule to remove two of its own sulfur atoms to donate to octanoic acid. Interestingly, this action renders lipoyl synthase unable to catalyze any further reactions. “In other words, the enzyme kills itself after it does a turnover,” Booker says. “It’s completely novel, and a lot of people didn’t want to believe this mechanism at the time.”
Another radical SAM enzyme called Cfr confers antibiotic resistance, most notably to some strains of Staphylococcus bacteria. Cfr adds a methyl group to the bacteria’s ribosomal RNA, which blocks antibiotic binding to the ribosome. Booker and his colleagues solved the three-dimensional structure of the related enzyme, RlmN, and elucidated the mechanism that Cfr and RlmN use to add that methyl group. Because the atom that receives the methyl group is inert and can’t easily accept new chemical groups, Cfr has to take a methyl group from a SAM molecule, strip it down, and then rebuild the methyl on the target RNA, rather than just transferring it whole. Now that he knows how Cfr works, Booker is looking at ways to stop the reaction and prevent antibiotic resistance.
Booker’s latest endeavor is a big one. He’s partnered with several other labs to assign functions to radical SAM enzymes. Of the protein family’s almost 115,000 members, the vast majority catalyze unknown reactions, so Booker and his team have their work cut out for them.
Granular Investigation
J. Paul Taylor is also interested in unknowns, only his relate to neurodegenerative diseases. In 2004, Taylor was just starting his lab at the University of Pennsylvania School of Medicine. A trained clinical neurologist with a love of research, he was interested in the genetic basis of motor neuron diseases but didn’t have a set research program. “I basically started my lab with no projects and just followed what came in the door at the clinic,” he recalls.
What came through the door were patients with odd versions of common neurodegenerative and muscle diseases: families with inherited diseases that don’t fit neatly into a standard diagnosis, or patients with genetic mutations who develop something other than the expected syndrome. Neurologists call these patients outliers – cases that can’t be explained by known disease-causing genes. “Those are the ones I went after, and we began to find a whole series of new genes that have given us complete insight into the basis of such diseases,” says Taylor.
By sequencing the outlier patients’ exomes, or expressed DNA, Taylor and his collaborators have been able to pinpoint the genes responsible for their symptoms. Surprisingly, he found evidence that many seemingly unrelated degenerative brain diseases are caused by mutations in RNA-binding proteins associated with so-called stress granules. These clumps of RNA and protein assemble when a cell encounters unexpected stress, such as extreme heat, and needs to quickly switch up the genes it’s expressing. By sponging up loose RNA, the cell can prevent the nucleic acid from being translated into protein until the stress is over. Taylor and his colleagues discovered that disease-causing RNA-binding proteins fall into two classes: those that are part of the granules themselves, and those that assist in granule assembly or disassembly.
Taylor has moved his lab to St. Jude Children’s Research Hospital in Memphis, and is now focusing on how these mutant RNA-binding proteins produce disease. He learned that the mutations can cause hyper-assembly of granules or impair granule disassembly. But the mutant proteins are also very prone to forming amyloid-like fibrils, such as the ones found in patients with amyotrophic lateral sclerosis or certain illnesses causing dementia. “We don’t know if the bad thing is the accumulation of these fibrils, or the fact that you are holding the granule together too long and the RNAs are not liberated to be used – or both,” he says.
Another puzzle Taylor wants to solve is why these ubiquitously expressed RNA-binding proteins cause disease only in neurons and muscles, and why mutations that exist throughout life don’t cause disease until a person is 40 or 50 years old. “These questions have been there since I was born, and for all I know, they may still exist when I’m long gone,” he explains. “But they’re always in the back of my mind every time we make an advance.”
Chromosome Segregation
In the late 1990s, Sue Biggins had mutants on her mind as well. She was a postdoctoral fellow in the lab of geneticist Andrew Murray, who was then at the University of California, San Francisco, and is now an HHMI professor at Harvard University, using a new technique to track yeast chromosomes. Looking through a microscope, Biggins noticed a group of mutant cells that couldn’t seem to segregate their chromosomes correctly during cell division. When she examined those mutants more closely, she saw that a chromosomal structure called the kinetochore was affected. “So I started digging deep in the literature to learn more about kinetochores, and that’s when I realized how little was known,” she recalls. When it came time to start her own lab at the Fred Hutchinson Cancer Research Center in Seattle, Biggins wrote a research proposal on the kinetochore and what she wanted to learn about its components, and how they function.
Kinetochores are complex molecular machines made of hundreds of proteins. They connect chromosomes to the long, thin microtubules that tug them to opposite ends of a dividing cell. But this is no easy task. Microtubules are constantly in flux, growing and shrinking at a rapid pace, and kinetochores need to keep their grip on them while simultaneously bearing the load of the chromosome. This interaction is so crucial to cell division that a surveillance mechanism called the spindle checkpoint immediately halts the cell cycle if a single kinetochore is not properly attached to its microtubule.
In 2000, just after opening her lab, Biggins decided that her best shot at understanding the kinetochore was to remove it from its complicated cellular milieu. She chose to isolate a relatively simple kinetochore, a complex of about 250 proteins from budding yeast. “It was very much trial and error and brute force, but my grad student figured it out,” says Biggins.
Her research quickly took off from there. With pure kinetochores in hand, Biggins made several discoveries about the molecular machines. When she and her University of Washington collaborator Charles Asbury added microtubules to the mix, they made the surprising finding that tension directly stabilizes kinetochore attachments to microtubules. As with Chinese finger traps, the harder the microtubules pull on the kinetochores, the stronger their grip on chromosomes. Her team has also identified many of the molecular events that regulate kinetochore assembly and the spindle checkpoint pathway.
In 2012, Biggins and Tamir Gonen, a group leader at Janelia Research Campus, published the first-ever three-dimensional structure of an isolated kinetochore attached to a microtubule. Their images revealed a key factor in the molecular machine’s ability to maintain its grip on those dynamic microtubules: multiple attachment points. “It’s like you’re climbing a rope and someone’s always pulling the rope out from under you,” says Biggins. “One way to stay attached is to have multiple contacts so that if one releases, the other one is still there.”
Ultimately, Biggins would like to understand what’s gone awry in cancer and other diseases that have the wrong number of chromosomes, such as birth defects. “Aneuploidy is one of the biggest hallmarks of cancer cells,” she explains. “Whether and how often it arises due to altered kinetochore function, no one knows.” To figure that out, she’ll have to isolate the human kinetochore. So far, no one has done it, but Biggins and her team are already working on the challenge.
A 3C Concept
Job Dekker has been trying to answer a different question involving chromosomes for almost 20 years. As a postdoctoral fellow at Harvard University, he started thinking about how genomes and chromosomes work from a different point of view. “Most people see chromosomes as long molecules full of information,” Dekker explains. “But I’ve always been driven by the idea that if we want to understand how cells work with that information, we have to understand how chromosomes are organizing, how they live inside cells. What does the living genome – the real chromosome – actually look like?”
What we do know is that the chromosome is extremely long and very dynamic. During mitosis, it needs to fold its six feet of DNA into an improbably compressed form. At other times, the very same chromosome must reorganize itself in a completely different, less compact, fashion to make its genes accessible for transcription. Both forms of DNA are physically distinct and extremely customized for the tasks at hand.
To determine what a chromosome looks like in three dimensions, Dekker developed a technique for detecting physical interactions between DNA segments. The result is comparable to a molecular microscope. “I remember when I proposed this in a lab meeting, I just had a rough scheme of how this would work. Almost everybody said it would never work,” Dekker recalls. But it did. In spades. Today, hundreds of scientists around the world are using Dekker’s chromosome conformation capture, or 3C, method to find connections between far-flung regions of DNA.
The concept behind 3C is surprisingly simple. Because DNA is folded, genes that are otherwise far apart along the linear molecule can end up extremely close together in three-dimensional space. By cross-linking neighboring areas of DNA and then cutting those regions into small pieces for sequencing, Dekker was able to decipher which parts of the chromosome interact with each other. The more interactions between segments, the more closely the segments are associated in space.
Since opening his own lab at the University of Massachusetts Medical School in 2003, Dekker has refined 3C to visualize entire genomes. In 2009, he published the first three-dimensional map of the human genome. The map revealed a lot about chromosomes, including the fact that loops of chromatin are used to activate the right genes at the right times, and that DNA is compartmentalized into “neighborhoods” of active and inactive genes. The map also provided physical evidence for how some genes can be regulated by distant bits of DNA called enhancers.
Although Dekker has finally created the map he dreamed of back in 1998, he is nowhere near ready to move on to a new problem. “I thought that if we solved the structure, we would get all the answers,” he admits. “We did get some answers, but it also raised a lot of questions that we didn’t even think of asking earlier.” Answers that he intends to pursue during the next five years, as an HHMI investigator.
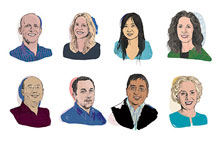